Stormwater Best Management Practices in an Ultra-Urban Setting: Selection and Monitoring
Monitoring Case Study-Modified Dry Pond BMP and Grassed Swale BMP, Charlottesville, Virginia
This case study is based on an evaluation conducted in two phases of a modified dry pond and grassed swale by Yu et al. (1993) and Yu et al. (1994).
In 1991, the Virginia Department of Transportation initiated a two-phase field study to test the pollutant removal efficiency of selected BMPs to obtain detailed information necessary to develop design guidelines for the stormwater BMP section in its Stormwater Management Manual. This study focuses on two BMPs: a dry detention pond located on the grounds of the University of Virginia near the intersection of Massie Road and Emmett Street, and a grassed swale located in the median strip of U.S. Highway 29 at the intersection with Hydraulic Road in Charlottesville, Virginia. The grassed swale operates by settling and infiltrating stormwater runoff from roads or highways along its entire length. The immediate drainage basin for the pond is a parking facility for daily commuters and athletic event traffic. The entire watershed contributing to the detention pond is approximately 3.2 ha (7.9 ac) in size, with 60 percent of the drainage area consisting of the paved parking facility.
Study Objectives
-
Evaluate the pollutant removal efficiency of a dry pond detention facility.
-
Evaluate the pollutant removal efficiency of a grass swale that collects runoff from an urban highway.
-
Recommend design guidelines for stormwater BMPs.
Design and Operation
Pond
The detention pond was initially designed and constructed solely for stormwater quantity purposes. Figures 51 and 52 show both the detention pond and parking area and a more detailed layout of the detention pond. The pond was sized to detain the additional post-development peak runoff flow for the 2- and 10-year storm events in order to release at the predevelopment conditions. No provisions were made for water quality improvements.
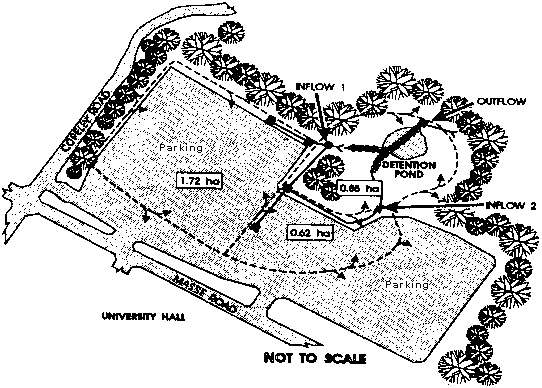
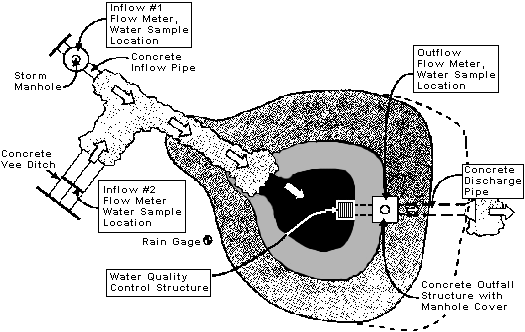
Extending the retention time of a dry pond is an effective and low-cost means of removing particulate pollutants and reducing the downstream effects of runoff such as stream bed and bank erosion. To create an extended detention dry pond, the outlet structure was modified to provide a slow release of the runoff from a designated storm (i.e., 2-year frequency or less). A three-sided concrete structure was cast around the inlet of the outfall pipe, with the sides of the open end of the structure grooved to allow insertion of plywood templates. For this study, the plywood template contained a 7.6 cm (3 in) diameter orifice; however, any type of orifice shape or size could be used in future studies. Figure 53 shows the outfall modification.
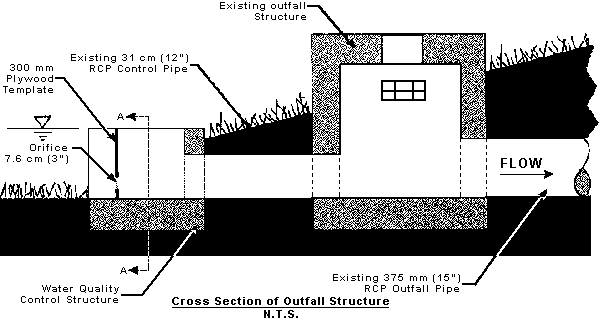
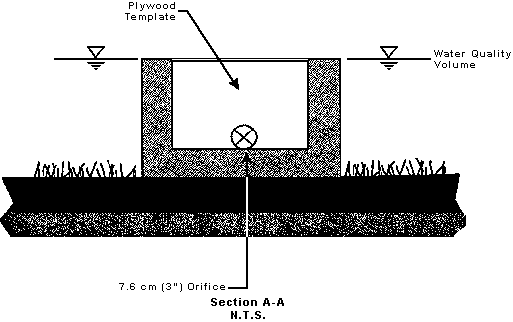
Swale
The grass swale is approximately 128 m (420 ft) in length and receives runoff from approximately 0.6 ha (1.5 ac) of drainage area. The grass in the swale is mowed on a regular basis, yielding an average grass height between 7.6 and 15.2 cm (3 and 6 in). No fertilizers are applied to the swale area. In Phase I, the swale was subdivided into four sections based on length and slope, with the entire length of the swale evaluated. Table 27 gives the section and slope information for the divided swale. In Phase II of the study, wooden barriers were constructed to limit lateral inflows into the swale so that pollutant mass balance estimates could be made more accurately (see Figure 54). In addition to this modification, only the lower 30.5 m (100 ft) of the swale were evaluated. Figure 54 shows the modified lower portion of the swale that was studied during Phase II.
Table 27. Swale section and slope data
Station (m) |
Drainage Area (ha) |
Longitudinal Slope (%) |
0 |
0.05 |
- |
33 |
0.11 |
0 m - 33 m: 3.2 |
68 |
0.19 |
33 m - 68 m: 3.8 |
100 |
0.25 |
68 m - 100 m: 6.5 |
Total |
0.6 |
|
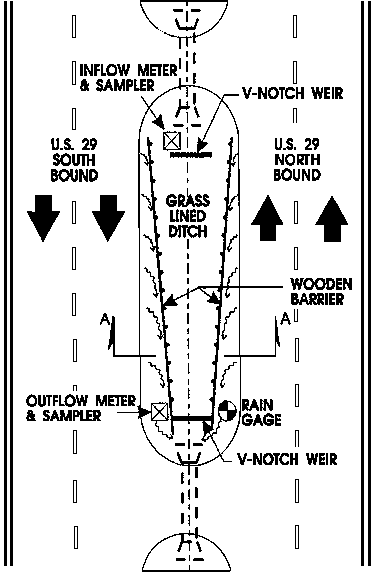
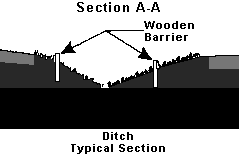
Monitoring Program
Pond
For the first phase of the study, data from seven storms were collected. For the first three storms, only the pollutant concentration was determined. The flow monitoring equipment was not yet assembled for measuring the corresponding flows into and out of the pond. The remaining four storms were monitored for both flows and concomitant pollutant concentrations. Of the remaining four storms monitored, the first two were a baseline study of efficiency only since the orifice was not in place; however, the last two storms were monitored with the extended detention orifice in place.
Rainfall data were measured using a Plexiglas wedge gage located at the site. The information was corroborated using a nearby rainfall gaging station. Flow was measured at all inflow and outflow points at the detention pond. Measurements were made using a conventional 90 degree V-notch weir and a portable Plexiglas weir designed to fit into a circular pipe. The V-notch weir was installed at inflow location 2, and the Plexiglas weirs were installed at inflow location 1 and the outflow (see Figure 51). Manual grab samples were taken at both the inflow and outflow at 15- to 30-minute time intervals during each storm event. Between three and seven samples were collected for each parameter. The parameters analyzed included total suspended solids (TSS), total phosphorus (TP), zinc (Zn), and particle size distribution (PSD). TSS and TP were measured for all storms; however, Zn was measured for the seventh storm only. Pollutant removal efficiency was calculated based on an average mass balance percentage removed. Table 28 gives the removal efficiency for the storms for which both flow and pollutant data were measured. A dash (-) entry indicates that removal efficiency data were not collected. Under the first phase of the study, the detention times for storms 4, 5, 6, and 7, were 1.5, 1.5, 3.1, and 3.2 h, respectively.
The data collection during Phase I appears to suggest that a detention pond with a short detention time of 1.5 h could provide a fairly significant amount of pollutant removal; however, it should be noted that storms 4 and 5 were low-intensity storms with low concentrations of pollutants. It is likely that the riprap conveyance channel to the pond and the low flow channel through the pond provided a mechanism sufficient to reduce the pollutant loads as they moved through the system. As Table 28 shows, once the orifice was in place, the removal efficiencies decreased; however, storms 6 and 7 were long-duration, high-intensity storms.
For the second phase of the study, analyses for chemical oxygen demand (COD) were added to the study. To reduce the need for chemical analysis, a rectangular weir was installed below the confluence of inflows 1 and 2 so that only one point needed to be monitored (see Figure 51). In Phase II, four storms were monitored for both flow and pollutant concentration. Water quality samples were taken using both manual grab sampling and automatic samplers. The detention times for storms 8, 9, 10, and 11 were 0.34, 1.55, 0.09, and 1.45 h, respectively.
Swale
For the first phase of the study, six storms were monitored from March to June of 1992. Water quality samples were collected for all six of the storms, but flow measurements were taken for the last four storms only. Therefore, only the last four storms were reported for this phase. Sampling stations were located at four lengths starting 25 meters from the edge of the asphalt at the intersection. Figure 55 shows swale cross sections at each of the four locations. Flow and water quality were measured at each station to determine a mass balance between inflow and outflow concentrations. The drainage area for each station was then used to determine a mass flux per unit drainage area. A normalization was needed due to the amount of untreated lateral inflow at each section of the swale.
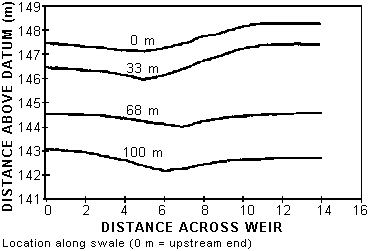
Flow was measured using 90 degree V-notch weirs. Depth was manually measured at each sampling time and converted to flow. Due to the low-flow conditions in the swale, the use of automatic flow meters was deemed impractical. Manual grab samples were collected as the water flowed over the V-notch at each station, with 500-mL grab samples taken during the first 30 minutes of the storms. Three to six samples were collected at each station for each storm. Total suspended solids (TSS), total phosphorus (TP), and zinc (Zn) were evaluated; however, zinc was evaluated only for the last storm monitored. Table 29 gives the pollutant removal efficiency for the four storms monitored. The percent removal efficiencies were determined using a mass balance method. A dash (-) entry indicates that data were not collected.
For the second phase of the study, five storms were monitored from November to July of 1993. Flow and water quality were measured to determine a mass flux. The drainage area for the lower 30.5 m (100 ft) portion was then used to determine a mass flux per unit drainage area. Because of the wooden barrier modification to reduce lateral inflow, normalization was not required (see Figure 53). Flow was measured using 90 degree V-notch weirs. Depth was manually measured at each sampling time and converted to flow. Automatic samplers were used to take runoff samples at timed intervals.
In addition to the pollutant parameters measured in Phase I, chemical oxygen demand (COD) was also measured. Table 29 gives the pollutant removal efficiency for the five additional storms monitored. The pollutant removal efficiencies were calculated using the mass balance method. The negative removal efficiencies given in Table 29 were caused by a higher flow leaving the swale than entering the swale because the mass flux equation used to determine removal efficiency is flow-dependent. During the first two storm events (7 and 8), the wooden barriers failed to prevent flow from entering the swale laterally. During the remaining period of monitoring, a plastic liner was placed along the lateral barriers to improve their performance.
Conclusions
-
A dry detention pond filled with a small outlet orifice can provide pollutant removal rates ranging from 30 percent for Zn to about 55 percent for TSS. The average overall pollutant removal efficiency was about 40 percent.
-
Detention pond storage volume and outlet structure are important design parameters. Riprap low-flow channels and vegetation at the pond bed help increase removal efficiency.
-
Detention time is usually considered to be one of the most important pollutant removal factors; however, based on the findings presented in this study, it appears that it is not the only determinant of pond efficiency.
-
Highway swales, when properly designed and maintained, can be cost effective in removing pollutants in highway runoff, especially for smaller and low-intensity, long-duration storms.
-
Swale length, longitudinal slope, and vegetation type are important considerations in swale design. Swale check dams may improve pollutant removal efficiency, but they get in the way of periodic mowing.
References
Yu, S.L., R.J. Kaighn, and S.L. Liao. 1994. Testing of Best Management Practices for Controlling Highway Runoff, Phase II. Virginia Department of Transportation, Report No. FHWA/VA-94-R21, Richmond, VA.
Yu, S.L., S.L. Barnes, and V.W. Gerde. 1993. Testing of Best Management Practices for Controlling Highway Runoff. Virginia Transportation Research Council, Charlottesville, VA.
|